The nanoscale world is the realm of the truly small. One nanometer is a billionth of a meter, about 100,000 times thinner than the sheet of paper on which these words are printed. If you could shrink to that height, atoms would be from ankle- to waist-high, and a single molecule would wiggle and jump as you watched an electron pass through. The ridges of an old 33-rpm vinyl recording would rise before you like mountain ranges (pictured on this page, several tracks from the Beatles’ “Eleanor Rigby”). You would quickly realize that objects and forces from your new vantage point are not just quantitatively smaller, but qualitatively different. Matter at that scale sometimes defies classical, Newtonian physics. Light is seen to be both a wave and a particle. Caged electrons tunnel through atoms-thin walls, releasing energy as they escape confinement. Proportionally, surface area becomes vastly larger and more important than volume, leading to significant changes in physical properties.
But if we can’t see these things, how can we understand them? No Small Matter: Science on the Nanoscale (Belknap/Harvard) illustrates in photographs and words the tools, concepts, and applications of nanoscience. The field is a thriving nexus of research (see “Thinking Small,” January-February 2005, page 50); at Harvard, no fewer than three centers and two institutes study different aspects of the nanosphere, engaging chemists, physicists, biologists, engineers, and even medical-school clinicians.
The new book—a sequel of sorts to On the Surface of Things: Images of the Extraordinary in Science (Chronicle Books, 1997; see “Phenomenal Surfaces,” July-August 1997, page 41)—is the second collaboration between photographer and research fellow Felice C. Frankel, who chose the images, and Flowers University Professor George M. Whitesides, a scientist with a way for words. Harvard Magazine presents a selection of images and observations from the book below.
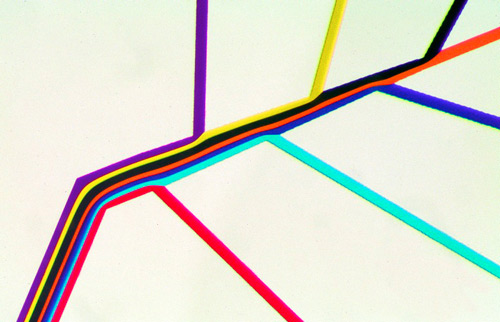
P.J.A. Kenis et al., “Microfabrication Inside Capillaries Using Multiphase Laminar Flow Patterning,” Science 285 (1999): 83-85
Laminar Flow
When confined to a tiny channel the width of a human hair, familiar fluids like water behave differently. If the flow is slow and the viscosity high, streams can meet, yet not mix. They maintain distinct layers, as if the fluids “were a sheet or slab rather than a liquid.” The intertia of the fluid itself, which at large scales leads to turbulence and mixing of streams, dwindles to unimportance, and the streams run parallel to each other. Blood in capillaries flows this way. “Arms of a glacier show the same behavior when they meet….Even people in narrow corridors move in an orderly stream; being close to a wall keeps everyone in line. If the corridor is wide, people meet, stop, chat, and swirl.” Microfluidic systems are “used in biotechnology to analyze blood and urine, to grow cells, and to crystallize proteins that are targets for new pharmaceuticals.”
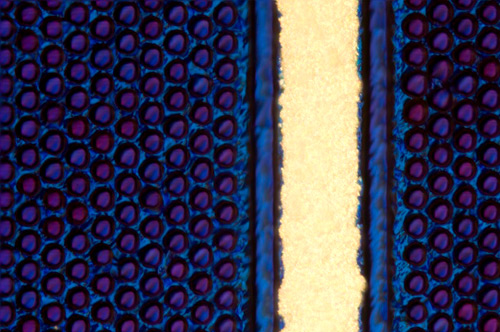
Photograph by Felice Frankel
Solar Cells
Solar cells, like this silicon wafer of light-absorbing hexagonal pits separated by a narrow metal conductor, generate electricity when particles of light (photons) knock electrons loose from a silicon layer. One photon bumps one electron, unless the photon is very energetic: then it might knock two electrons free. A solar cell is made of two silicon layers: one has extra, “free,” electrons; the other is missing electrons, known as “holes.” Sunlight hitting free electrons makes them take a one-way jump to the other layer—which they want to do anyway, to form an electron-hole pair—a match made in heaven, so to speak. “If everything works (and it usually doesn’t), the itinerant electron may return home by going through an external wire, and turning a motor or generating hydrogen along the way…,” notes Whitesides. But capturing energy from sunlight, even when it is abundantly available, is not cheap. “Solar electricity is a good idea, but not a good enough idea to save us from ourselves,” he says. “Either we have to find more energy elsewhere, or use less.”
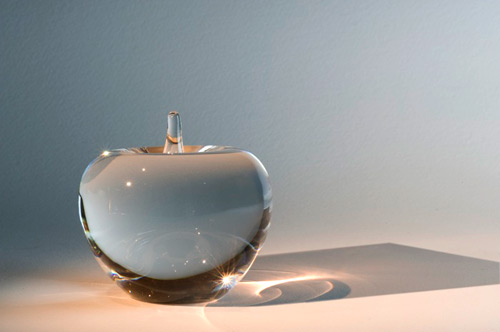
Photograph by Felice Frankel
The Quantum Apple
How can a glass apple cast a square shadow? The answer is simple: it cannot. Frankel has combined the shadow cast by a cube with that of the apple (for an explanation of how the photograph came to be, see the online extra, “Notes from the Photographer”). The image is a metaphor for the seeming contradictions common in quantum physics. The most familiar of these, that light is both a wave and a particle, appears as a concept several times in No Small Matter. When light is moving, it is a wave. When it hits something, it is a particle. “Even you and I are waves,” writes Whitesides, “albeit waves with crest to crest distances of 10 to the minus 35 centimeters (a distance so small that it is unimaginable).”
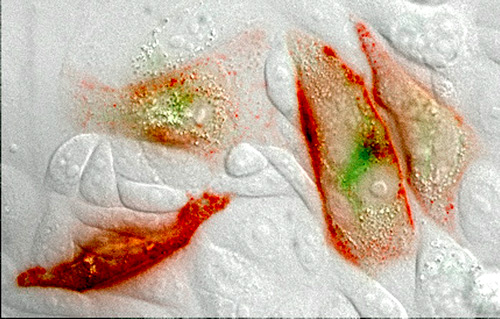
Photograph by Felice Frankel
Quantum Dots
A quantum dot is like a cage for electrons. Scientists build the cage as a semiconductor sandwich, in layers: a conductive layer where the electrons live, pressed between two layers that act as insulators to prevent electrons from escaping. Then they snip away until only a dot of material is left. Quantum dots are so small that their size determines how many electrons they can hold. Because each electron has a fixed charge, a dot’s energy is quantized—it changes only in whole steps (1,2,3…), according to the number of electrons it contains. Ultraviolet light excites the electrons in a quantum dot so that they emit visible light in a predictable way. Small dots with few electrons emit blue light, large ones emit red. In biology, quantum dots can be used like dyes. “In Thailand, at night,” Whitesides recalls, “fireflies collect in particular trees, and their flashing—sometimes astonishingly synchronized—shows the shape of the tree and the structure of its branches.” Here, quantum dots that have been superimposed on a microscropic image of cells, “like nanoscopic fireflies,” illuminate the structures within.
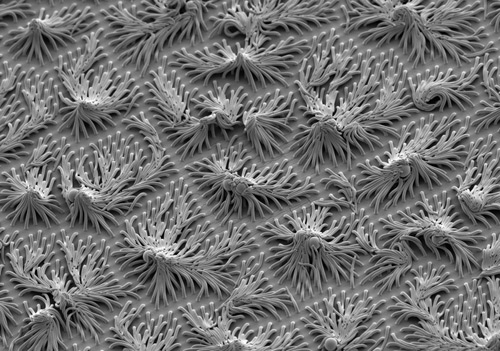
Image by Joanna Aizenberg, Harvard University. B. Pokroy et al., "Self-Organization of a Mesoscale Bristle into Ordered, Hierarchical Helical Assemblies," Science 323 (2009): 237-240
Capillarity
Polymer tendrils one thousandth the width of a hair appear to cling to these small polymer spheres. Why? The explanation lies in the one thing missing from the photograph: water. The spheres once floated, suspended in the water, while the fronds waved below. “As the water evaporated, the spheres settled on the fingers, and capillarity—the tendency for the last thin film of water to form beads rather than to spread—pulled the fingers to the balls.” Water molecules are attracted to each other more so than to other molecules, and so form beads because that is a shape that allows them to bond most efficiently.
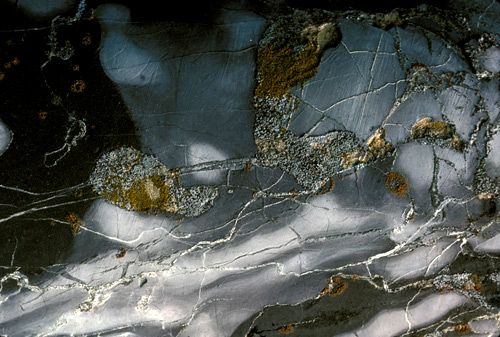
Photograph by Felice Frankel
Cracks
Over eons, lichens such as these can crack and reduce rock to rubble. But what is a crack? Following it to its terminus at the nanoscale, does a crack propagating in a piece of glass rend even molecules, breaking bonds between atoms? Sometimes, yes. Existing models of the way things crack postulate such an atomically sharp tip. But experiments suggest otherwise. Scientists use multiscale modeling to understand fractures across different scales of length and energy, but at the tip, and on the smallest scale, their models break down. “A crack smaller than an atom makes no sense,” writes Whitesides, “but how matter progresses from two distinct pieces to one is not obvious at the atomic scale.”
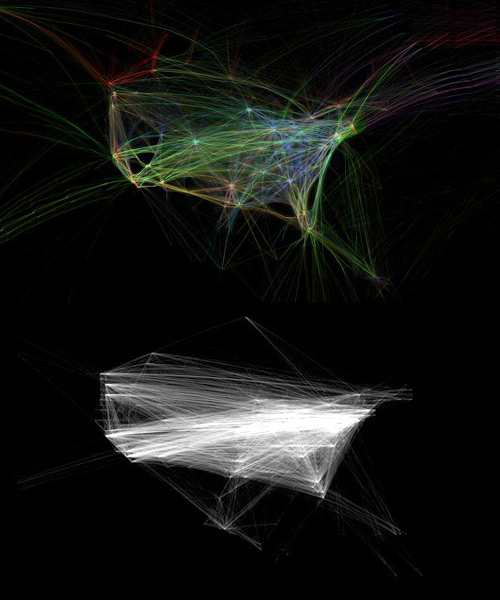
Top: Aaron Koblin; Bottom: Chris Harrison, Carnegie Mellon University
The Internet
Comparing maps of communication past and present reveals parallel patterns. The upper image shows the movement of passengers on airplanes across the United States; the lower one, the flow of bits across the Internet. “It is the largest thing we have ever built,” says Whitesides, “and we have assembled it from transistors—the smallest things we know how to make. It is a chrysalis we are forming around the planet…a table where we sit to gossip, a suq where we buy and sell; a shadowy corner for planning mischief; a library holding the entire world’s information; a friend, a game, a matchmaker, a psychiatrist, an erotic dream, a babysitter, a teacher, a spy….The best and worst and most ordinary of us reflected—and perhaps distorted—in a silvery fog of bits.”
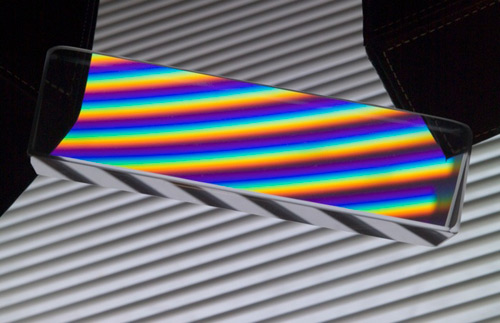
Photograph by Felice Frankel
Prism
A prism reveals the wave-like nature of light. Visible light seems colorless until the prism reveals the rainbow of its constituent parts, with the colors corresponding to different wavelengths. The glass disrupts all the wavelengths, but not equally. When light enters a material, it changes speed according to the material’s refractive index. But glass is a special material in which the refractive index changes based on the wavelength of the light. In glass, high-frequency violet light slows more than red light. When, in addition, a wavelength of light enters glass at an angle, the wave bends around the point that enters the glass first and slows first. Light that slows more—like violet—bends more, and consequently follows a different path through the prism and out of it. White light becomes “a spectral peacock’s tail—red, yellow, green, blue, violet—as it bends through a prism.”