Your mother has had difficulty walking, and this morning she is slurring her words. Concerned that she might have had a stroke, you rush her to the hospital. Doctors there have a different theory: she might have ALS (amyotrophic lateral sclerosis), a disease of the nervous system, specifically of the motor neurons that control the muscles. Without treatment, she would have three to five years to live.
A nurse takes a biopsy to collect some of her skin cells. A laboratory technician quickly cultures some of them in a petri dish, then adds a chemical cocktail that directly reprograms the skin cells, turning them in a single step into motor neurons. These transformed cells, identical to your mother’s motor neurons, can now be easily tested for the known defect associated with ALS.
The test confirms the diagnosis. The doctors have a drug that will prevent or slow progression of the disease, but the treatment is toxic to the heart muscle in 20 percent of patients, so more tests are required. During the course of a week, using the same skin cells, physician-scientists use another chemical cocktail to create an induced pluripotent stem cell (iPS) that is capable of producing genetically perfect copies of every cell in your mother’s body. Then, applying what science has learned about developmental biology, they use chemical signals to guide the iPS cell, in a series of divisions, to differentiate into cardiomyocytes (cells of the heart)—creating a thin layer of them that actually beats in a petri dish. Now they can test the drug for toxicity against your mother’s own heart cells. The cardiomyocytes keep beating, so she can safely take the drug. It is time to go home.
In reality, deriving an iPS cell line takes as long as a year, and there is still no cure for ALS. But this scenario illustrates the tangible progress scientists have made in using stem cells as tools: to change the state of cells; to understand disease mechanisms; and to speed drug discovery. Many of the pathbreaking discoveries in the field—directly reprogramming defective motor neurons caused by ALS, drugs that halt or slow cellular degeneration in the lab, and the beating layer of heart muscle—are real, and were made by Harvard scientists. Stem-cell researchers are even beginning to learn how to harness the body’s own repair mechanisms to promote healthy aging (see “A Hidden Youthfulness,” page 27).
Research on stem cells, once hailed primarily for the promise of cell-transplant therapies, is flourishing in new areas even as progress toward that initial goal continues (see “Stem-Cell Science,” July-August 2004, page 36). “We have not given up on the goal of transplanting cells back and repairing the body,” says Cabot professor of the natural sciences Douglas Melton, co-director of the Harvard Stem Cell Institute (HSCI; www.hsci.harvard.edu), “but this new aspect of using stem cells as tools for drug discovery has got us really excited. And that could lead to the first products, using stem cells as tools to find…drugs that slow degeneration.”
Two HSCI “core facilities” in particular have turbocharged the pace of discovery. These specialized laboratories—one devoted to creating new disease-specific stem-cell lines, the other to screening chemicals in order to advance human therapies—act as centers of expertise for scientists throughout the University. Each is itself a scientific instrument, as well as a constant source of new tools.
The Ethical Stem Cell
Reprogramming adult cells to become “pluripotent”
Chad Cowan, tall, laid-back, and lean, leads the iPS core facility. The first induced pluripotent stem cell, he explains, was created in 2006 when Shinya Yamanaka of Tokyo University demonstrated that it was possible to reset mouse skin cells to a “pluripotent” state—in which they could become any cell in the body. The advantages of transferring this approach to human beings were clear. First, the technique raised the possibility that the ethically complicated human embryonic stem cells (HESCs) harvested from days-old embryos might no longer be needed. (To date, this has not proven true.) And second, as HSCI scientists immediately recognized, the technique also made it possible to create stem-cell lines—an infinite source of cells—from individual patients with specific diseases. (HESCs are not as useful for studying disease, because the embryos from which they come are presumed healthy.)
Having a renewable source of diseased cells in a lab, says Cowan, an assistant professor of medicine and of stem-cell and regenerative biology, “is like having a little human in a dish. You can ask all the same questions about human biology and human disease that you could ask of an actual human patient, only better.”
George Daley was among the first scientists to report reprogramming human iPS cells, Cowan continues. An associate professor of biological chemistry and molecular pharmacology and of pediatrics at Harvard Medical School (HMS), Daley created cell lines representing 10 different kinds of illness, ranging from “bubble-boy” disease to Parkinson’s; today, he chairs the steering committee for the iPS core. Meanwhile, his colleague Kevin Eggan, an assistant professor of stem cell and regenerative biology in the Faculty of Arts and Sciences (FAS), derived motor neurons from a patient with ALS. Science magazine called the reprogramming of cells the breakthrough of the year for 2008.
Even so, the derivation of pluripotent cells has not been perfected, Cowan explains. To create the first iPS cells from mice, Yamanaka used a combination of four retroviruses that became integrated into the genome of the cell, leaving it slightly different genetically from the original cell. Even worse, the technique involved cancer-causing oncogenes, which meant that anyone hoping to use the discovery in human therapies would need to develop a better approach. A subsequent technique developed by Konrad Hochedlinger, an HMS assistant professor of medicine and of stem-cell and regenerative biology, has eliminated the cancer-causing genes by substituting a different type of virus. “The new technologies are trying to get away from viruses,” says Cowan, “because they integrate into the host genome and could interrupt essential or important genes. The iPS core to date is still using a viral-based system, but after you create the iPS cells you can remove it, leaving behind in essence an unmarked genome.”
Various labs, Cowan reports, are pushing further, working to replace the genes involved in the stem-cell reprogramming process with “chemical compounds that essentially do the same thing.” Eggan, working with HSCI director of translational medicine Lee Rubin, “has shown that it is possible to replace individual genes with chemical compounds,” Cowan explains. “Now it is just a matter of finding the right chemicals that work in combination with one another.” Adds Daley, who also directs the stem-cell transplantation program at Children’s Hospital, “Lots of people within HSCI are working on figuring out more efficient and more effective protocols for reprogramming.” Daley himself is developing new ways to make iPS cells from marrow and blood in addition to skin. “Chemicals are one cutting edge,” he says. “Identifying pathways”—the sequences in which cells convert one type of signal to another—“that contribute to or stand in the way of reprogramming is another.”
For now, the iPS core facility serves two goals. One is to culture, store, and distribute iPS cells already developed by HSCI to researchers worldwide. “We did it as a service for the community,” says Daley, “and I think it has been an important one. We have gotten hundreds of requests from labs to receive the lines.” (There are currently 14 lines available, with 10 more coming in early 2010.) The second objective is to assist Harvard clinicians and scientists who have unique patients afflicted with diseases they want to study using iPS cells. Generating such customized cells requires “a fair amount of expertise,” says Daley, “and the iPS core has it.”
“The great ambition, the dream” of the iPS core, says Cowan, would be to “make a stem cell for every patient who walked into a Harvard-affiliated hospital.” Daley, whose own work focuses on blood diseases, has a similar vision. The long-term goal of his lab is “to try to couple personalized stem-cell creation with blood regeneration and engraftment”: essentially, obviating the need for bone-marrow transplants by using the iPS platform to put healthy blood stem cells back into patients. This approach would eliminate the immune-rejection problems that come with marrow transplants, which often rely on multiple donors.
The challenge is that the patient’s own genes would still carry the genetic defect that caused the disease in the first place. But Daley has already demonstrated in mice the efficacy of doing gene repair in a petri dish. One of the advantages of this approach, he says, “is that you could precisely repair gene defects without disrupting other genes,” and prove it before generating specific cells, such as adult blood stem cells, that could be transplanted into a patient. Such a therapy would be “potentially curative—and safe.”
Although such personalized approaches are not yet practical, in part because creating and characterizing a new iPS cell line still takes from six to 12 months, that may not be true three to five years from now. In the meantime, with the technology still in its infancy, one of the steering committee’s challenges is prioritizing proposals for making new iPS cell lines. Study projects involving Huntington’s and Parkinson’s disease and schizophrenia were “among the most mature” proposals initially, says Cowan. Coming soon are new cell lines for polycystic kidney disease and type 1 diabetes.
Having iPS cell lines for various illnesses puts a powerful tool in the hands of researchers who may not know much about stem cells, but who are experts in particular types of disease, he says. For example, iPS cells will allow researchers to compare genetic and nongenetic forms of degenerative conditions, perhaps pointing to common pathways between the two forms of illness. Such pathways could become the target of drug therapies. And iPS cells also allow researchers to watch a given disease unfold. By the time many patients see a doctor, their illnesses are already full-blown, making it next to impossible to determine how they started. The iPS cells will allow researchers to watch, over and over, how diseases progress, so they can test ways to intervene. One day, iPS cells might even enable a full-scale clinical trial in a petri dish.
Cowan’s own research on adipocytes (fat cells) illustrates how iPS cells could accelerate research into the causes of obesity. He seeks to learn why, for example, some people can eat everything and remain rail-thin, while others gain weight on a modest diet. If there are genetic predispositions to obesity, clearly they interact with environmental and behavioral factors. Studying cells in a petri dish allows Cowan to control for those nongenetic variables. “You can just say, ‘Oh look, this fat cell doesn’t utilize lipids in the same way this normal fat cell does,’ and ‘Can we fix that by, say, giving it a chemical compound that will mimic the normal function?’ ”
Using iPS cells also lets him perform experiments at a scale that wouldn’t be possible if he had to rely on human subjects—liposuction patients, for example—for all the cells he needs. “We need to make thousands or even millions of fat cells if we want to screen chemical compounds for their effect on fat,” he explains, and iPS cells provide a “reliable, renewable source.”
Conditions like obesity and cardiovascular disease also involve multiple cell types signaling to each other: hypothalamic neurons communicating with fat cells in the case of obesity, or liver cells, fat, and vascular endothelial cells in the case of heart disease. To study complex interactions like these, Cowan needs all these cell types, all derived from the same iPS cell. Such combinations of human cells could then be studied in vitro or transplanted into mice so their interactions could be studied in a mammal model.
The Road to Treatments
Chemical screening to find new tools, pathways, and drugs
In the quest for new tools like iPS cells, says Douglas Melton, it is important not to lose sight of stem-cell science’s long-term goal: making cells to transplant into people. For that, the mammalian embryonic stem (ES) cell is still key, he says, because it is the only one that has not been genetically modified. Thus, even as HSCI works to create a bank of iPS cells for global distribution, it continues to maintain the world’s largest bank of ES cells, created at great expense with private money during the years when the second Bush administration suspended federal funding for the study of such lines. After the Obama administration reversed that policy last March, scientists anticipated that the Harvard lines would be approved for use in such research. Only a few of them have been. Harvard continues to maintain separate laboratories for many of its embryonic stem-cell lines—at considerable expense—while the NIH [National Institutes of Health] reviews their eligibility for federal funding.
Beyond the political obstacles, scientists still haven’t solved the problem of how to direct the process of differentiation in either ES cells or iPS cells to make specific cell types. Recapitulating the normal development program in a petri dish has proven extemely complicated, Cowan agrees. A protein signal that has a certain effect at one stage of the process—guiding an ES cell to become, for example, one of the embryonic “germ layers” such as endoderm (from which the gut, liver, and pancreas develop)—might have an entirely different effect at a later stage, or in a higher concentration, or within a different environmental niche in the body.
One way Melton has dealt with this problem is to do an end-run around the whole process. Instead of resetting a cell to a pluripotent state and then trying to control the ensuing cascade of cellular divisions to create a particular type of cell, he attempted to change one adult cell type directly into another—in a living animal. In 2008, using three transcription factors (a class of genes known to regulate cell fate during early development), he and postdoctoral fellow Qiao “Joe” Zhou succeeded in transforming a common type of pancreatic cell in mice into insulin-producing beta cells. This was a stunning achievement in what is now called direct reprogramming, the process of determining which genes are turned on and off in the cell. And because Melton derived the cells from a related cell type, only three transcription factors were required.
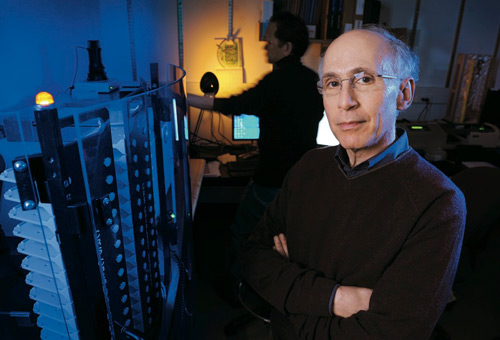
Photograph by Fred Field
Lee Rubin
But to duplicate this feat in humans so that it might one day become useful in treating diabetes, Melton will need to identify a drug, or combination of drugs, that will do the same thing. That is where HSCI’s second major scientific instrument—the therapeutic screening center, run by Lee Rubin—comes into play.
In fact, Rubin is directly involved in many of HSCI’s most important “translational studies”—moving basic research findings toward implementation in medical practice. Finding chemical means for reprogramming and directed differentiation is just one of his important roles. “He is a linchpin,” says Melton, “because without him, HSCI is, in a sense, just a basic-science department.” Rubin, Melton notes, is “an extraordinarily accomplished research scientist” who worked for a biotech company but has been brought back to the University to advance HSCI’s mission of curing disease. “All of the basic science we do here is going to lead either to our making cells that we can put back into people—and that indirectly involves Lee—or we are going to find drugs that block degeneration, or harness the body’s own mechanisms of repair by activating stem cells, and that leads directly back to Lee,” Melton explains. “We need to clone him.”
Rubin got his start in biotech working on a signaling molecule called “hedgehog,” a powerful reagent that scientists discovered caused certain cancers to grow more quickly. Rubin reasoned that seeking a countersignal that would reduce hedgehog signaling might stop or slow cancer, so he set up a screening system to find such an agent. His quest eventually led to the discovery of a promising drug that mimicked the body’s own mechanism for inhibiting hedgehog. The drug (now a Genentech property in phase II clinical trials) treats medullablastoma, a childhood brain cancer, and basal-cell carcinoma.
As it turned out, another agent that promotes, rather than inhibits, hedgehog signaling was already widely used by stem-cell scientists as part of a “differentiation cocktail” that could turn ES cells into motor neurons. It occurred to Rubin that he could make billions of motor neurons from ES cells to study disease in vitro, something that had never been done before. While he was still working in industry, a grant from the Spinal Muscular Atrophy Foundation enabled him to begin studying that disease and combine his newfound ability to make huge numbers of motor neurons with his screening system in order to find a therapeutic agent. “When pharmaceutical companies screen, they usually use cells that are easy to grow and easy to make in large numbers,” he explains. “Those are never neurons.” His research was the first to run a large screen using a neuron with disease characteristics: in other words, the first “disease relevant system” to identify therapeutics.
“In biotech,” says Rubin, a lively, fast-talking fellow, “the faster you can do things, the better it is.” While most stem-cell scientists remained focused on cell therapies, which can treat a small number of diseases, Rubin hit upon the notion of a real expansion of stem-cell use: to make cells involved in many different diseases. At the time, this involved the cumbersome technique of replacing a human ES cell’s nucleus with the nucleus of a cell from a diseased patient. Getting the embryos, the approvals, and the money to do this was not easy. The discovery of iPS “jet propelled” the idea by making the creation of disease-specific stem cells much easier. And it raised a significant possibility: that stem cells might be used to both speed up, and lower the cost of, drug discovery.
Developing a drug, including research and testing in human populations, typically takes five to seven years, and another six months to two years to secure regulatory approval. In the case of diseases affecting relatively small numbers of people, the costs can be prohibitive. “Suppose you want to study ALS,” Rubin says. You must test therapeutics for side effects, and “under the old system,” he points out, “to find an effect on a small population, you have to run a large trial. But if you have a disease like ALS where you don’t have that many patients, you can’t do many large trials.”
But by using iPS cells, that problem can be tackled from the other direction—by making iPS cells from hundreds or thousands of ALS patients over time, and then differentiating those cells to make motor neurons to identify patients for whom the drug might be effective, as well as cardiomyocytes and hepatocytes (liver cells), the two cell types most often involved in side effects from drugs. That way, potential therapeutics could be tested against a wider array of cells before anything goes into a person, Rubin says. “This offers at least the prospect of changing the way drugs are developed, in the sense that you would have better approaches for identifying drugs against particular diseases and for testing their safety. So that by the time you test it in a person, if all these things align, the clinical studies are smaller and more likely to be successful.”
This big idea—“It is really breathtaking”—might turn out to be true, Rubin says, though it will take years, and “actually finding better drugs that work in people,” to know.
Rubin’s therapeutic screening center allows him to screen more than 30,000 compounds and controls at a time. One of the ways he “adds value” to HSCI, as he puts it, is by screening for better drugs. “One of the things that stands between this big idea and reality,” however, is differentiating disease-specific iPS cells into particular cell types so that large-scale screens can be run against them. “We can make motor neurons, but we can’t make beta cells. There aren’t that many cells we can currently make right now.”
Rubin has therefore begun collaborations with numerous labs around Harvard to run screens for compounds involved in determining what tissue type the cell becomes. The center uses two approaches. The first employs previously identified factors present in normal development. The second proceeds blindly, saying, in effect, “We don’t know how to do it, but we can recognize it when it happens.” Robotic imaging devices allow center researchers to test, for example, for the presence of proteins known to be associated with various stages of cell differentiation. “Instead of one postdoc in Doug [Melton]’s lab testing a hundred things—whatever number is reasonable for a person to test,” Rubin points out,“we could test 30- or 40- or 50,000 variations of different kinds of molecules or combinations of molecules to see if any of them work at all.”
Rubin and Eggan just published a paper in which that kind of screening was used to find a drug to replace two of the genes traditionally used to make motor neurons. They might be able to replace all of the genes in the next year or two, Melton says. “Lee’s powerful new approach has been working spectacularly well.”
In January, Cowan and the iPS core facility will move from a location near Massachusetts General Hospital to the Cambridge campus, right next door to Rubin’s therapeutic screening center in the Sherman-Fairchild Biochemistry building on Divinity Avenue. The synergies are obvious—and Cowan and Rubin already collaborate. Harvard is among the very few institutions with both this chemical-screening capability and expertise in induced pluripotent stem cells, says Cowan, “so putting the two together is enormously powerful. And then the last ingredient—what makes this the most delicious cake ever, scientifically—is that we have some of the finest research hospitals on the planet. Their understanding of disease and patient phenotypes informs which stem cells we should make, and once we have them, what we should be doing to search for defects.” It is a triumvirate that Cowan believes will “give us the opportunity to make discoveries in human disease” that heretofore weren’t possible.
“Everything we thought stem cells would be good for, still is true,” says Melton. “Now we just have to get treatments into people.”