At Harvard, experiments involving mosquito sex are the purview of Flaminia Catteruccia, director of the insectary at the Harvard Chan School of Public Health. She is often consulted about mosquito-borne diseases such as malaria, dengue fever, and chikungunya, which together infect almost 600 million people worldwide each year. Most recently, she has called for genetic analysis of the Zika virus, also carried by mosquitoes, which has been linked to children born with small heads and underdeveloped brains. But what sets her apart from other scientists in her field is her genetic work on the mosquitoes that spread these diseases.
Within the insectary, locked behind multiple layers of biocontainment, the heat and humidity are reminiscent of the tropics in high summer. Stacks of mosquito-filled cages made of white mesh line the walls, loosely covered with clear plastic to keep the moisture especially high. Although wild mosquitoes are extraordinarily fecund, lab mosquitoes are somewhat deficient in the sex department, Catteruccia explains, as a doctoral student, having snipped the head and legs off a male mosquito, dangles the corpse from a pair of tweezers above a female, wings pinned akimbo in a petri dish. “This lucky fellow,” the student remarks, “is going to get his genes passed on to the next generation.”
Lab-bred male mosquitoes, it turns out, aren’t always this fortunate. Wild females typically won’t mate with them. Researchers learned this during field experiments: they released sterilized males into the environment, hoping that they would mate with females, who would then fail to produce offspring, leading to fewer mosquitoes in the next generation. The strategy has worked well with other insects, but not mosquitoes. In the wild, mating “happens in flight, in swarms,” Catteruccia explains. “There’s a lot of male competition for females.” And male mosquitoes raised in a lab, even from wild eggs, she says, can’t compete on the sexual battleground. If a female has to choose between a lab-released male and a field male, “she will know exactly how to go; but we still don’t know what makes a male a male in the eyes of a female.”
For researchers and public-health officials who hope to control mosquito populations, this acute preference is a potentially severe stumbling block in their efforts to build and disseminate safer, genetically modified mosquitoes. Yet research is steadily and rapidly nearing that goal, and the implications for success have prompted some scientists to raise significant ethical concerns.
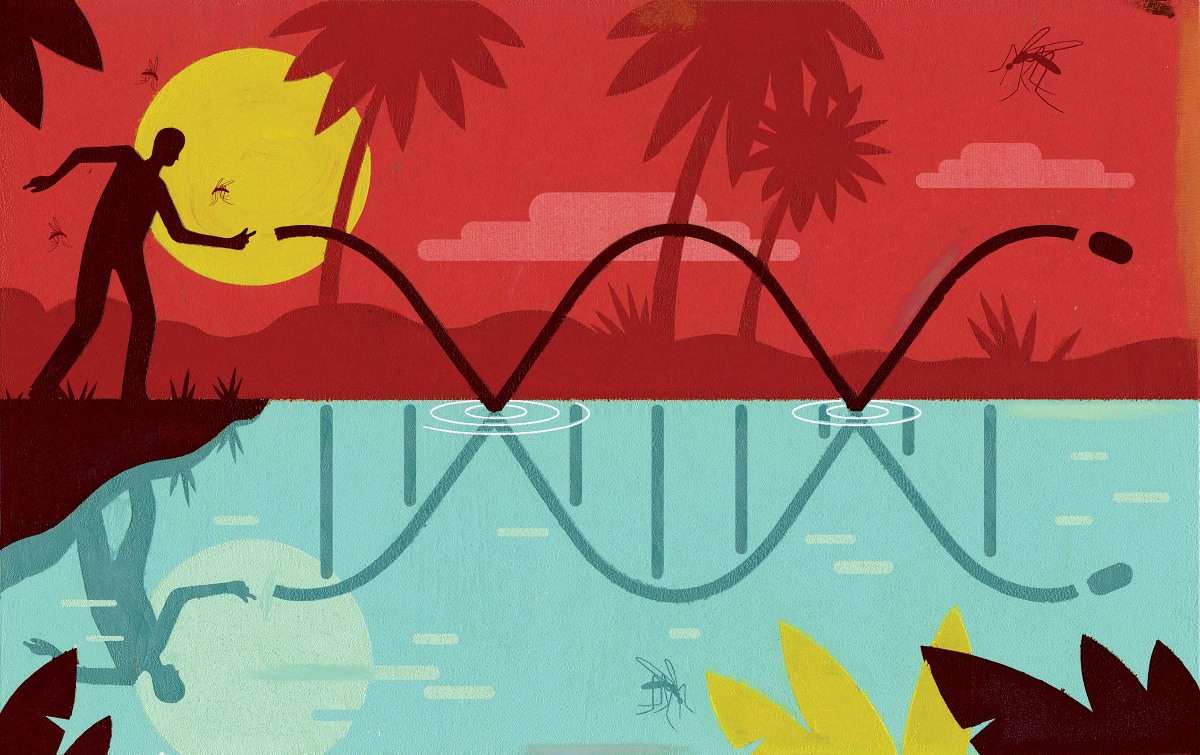
Illustration by Pete Ryan
Breeding a Better Mosquito
Imagine a mosquito genetically engineered so that it could not carry Plasmodium falciparum, the single-celled malaria parasite. (Research on the parasite’s genome is the subject of “An Evolving Foe,” March-April 2010, page 42.) Certain mosquitoes already have some immunity to P. falciparum—and permanently introducing that trait into wild populations of the mosquitoes that carry the disease and enhancing it, might prevent 200 million human cases of malaria, and save 600,000 lives, every year.
But the toll in human life doesn’t capture the full impact of malaria (detailed in “The Landscape Infections,” November-December 2001, page 42). Three billion people—nearly half the world’s population—live in areas where the disease is rampant. That has powerful economic effects. The burden on health systems alone is immense, Catteruccia points out. The disease also leads to reduced productivity, as well as loss of foreign investment. For already impoverished nations, she says, malaria is a poverty trap from which escape is difficult. And in Europe and North America, she says, the disease potentially could spread again, along with new threats like the Zika virus, because the climate is warming, and “insecticides are not as effective anymore, because mosquitoes adapt.”
Now an associate professor of immunology and infectious diseases, Catteruccia began studying mosquitoes as a graduate student in Italy, where “malaria was prevalent until the Second World War.” Though initially trained as a chemist, she joined a project studying mosquitoes and successfully adapted genetic techniques developed for use in lab animals to the insects. It was a first, but solving technical problems in research wasn’t her main interest, so she switched fields, ultimately earning a Ph.D. in molecular biology in order to focus on biological questions.
At Harvard, she began creating “knock down” insects, in which the function of a particular gene is dialed back—but not eliminated. One gene might control wing growth, for example, and mosquitoes with a modified version would be unable to fly. By knocking down genes and observing the effects in this way, she has been mapping mosquito genes to their biological functions, identifying those most important in development and reproduction.
More recently, advances in gene editing technology have allowed Catteruccia to create true “knock out” mosquitoes, in which a particular gene’s function is completely eliminated; this is now the state-of-the-art approach. Knockouts have been commonplace in standard lab animals such as mice for a long time, but their use in mosquitoes is new, and is a main focus of her lab. “Now we can generate stable mosquito lines that have [a particular] property, so you can study lots of mosquitoes at the same time and have reproducible results,” she explains. “It has really revolutionized mosquito research.”
The new tool that has made this possible, Crispr-Cas9, enables researchers to easily and inexpensively make precise edits to the genomes of a wide range of organisms (see “Speaking Nature’s Language,” page 55). But it has also opened the door to a previously unthinkable prospect: the possibility of editing the genes of entire species of mosquitoes, or any other sexually-reproducing animal or plant in the wild, potentially conquering plagues like malaria.
Gene Editing Untethered
When Andrea Smidler, one of Catteruccia’s research assistants, decided in 2013 to pursue a Ph.D., Catteruccia encouraged her to rotate into the labs of other scientists as part of her training. One of those scientists was George Church, Winthrop professor of genetics at Harvard Medical School. In Church’s group, Smidler met a young postdoctoral fellow, Kevin Esvelt, who had just had a revelatory thought: that Crispr-Cas9 could be used to create a gene-editing tool that could propagate beneficial traits through wild populations of any organism. Malaria could be eliminated. Mosquitoes could be wiped out in places like Hawaii, where they weren’t native and are spreading avian diseases, driving certain birds to ever-higher altitudes—and to the brink of extinction. Invasive plants could be tamed, corals modified to resist bleaching caused by warming seas. The possibilities seemed endless.
The tool Esvelt was describing is called a gene drive. In sexual reproduction, offspring inherit two versions of every gene, one from each parent. Each parent carries two versions of the gene, as well, so chance normally governs which particular variant of the gene will be passed on. But a gene drive ensures that one gene variant will win the lottery of life virtually every time and will almost always be passed on.
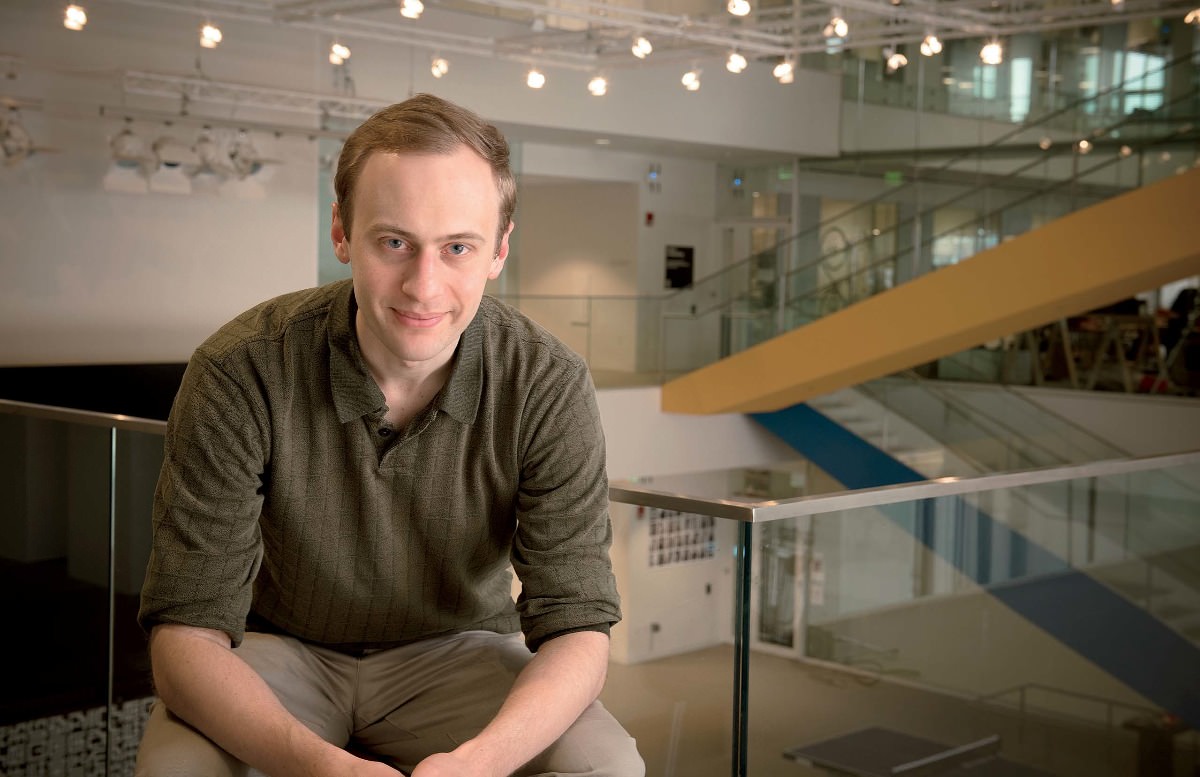
Kevin Esvelt was the first to realize that a new DNA-editing technology could let humans intervene in ecosystems by using genetic tools.
Photograph by Stu Rosner
Church, a genetics and genomics pioneer (see “DNA as Data,” January-February 2004, page 44), remembers seeing a naturally occurring gene drive for the first time while he was a graduate student at Harvard in the 1970s. His collaborator, Bernard Dujon, found that when his yeast carried one particular gene variant, all its offspring from all matings would, too. “We didn’t know what we had at first,” Church recalls, but “clearly, this element was spreading” fast with each new generation. Eventually, what the researcher had uncovered became clear: molecular machinery (called Meganuclease) that enables a particular gene variant to be inherited with relentless certainty.
The general public was just becoming aware of the possibility that genes might be subject to the same evolutionary pressures as individual organisms. In 1976, evolutionary biologist Richard Dawkins had published a controversial book, The Selfish Gene. Darwin had argued that evolution acts on individual organisms, and by extension on species, but Dawkins focused on genes as the level where evolutionary dynamics play out—survival of the fittest genes. The distinction Dawkins makes might at first seem purely semantic: the best genes are in the fittest individuals and both are more likely to survive and reproduce. But across many generations, as genes are mixed and remixed through sex—so that great-grandmother’s intelligence and great-grandfather’s keen eyes appear in countless numbers of their descendants—the idea of focusing on genes as the unit under selection makes a little more sense. Genes that are passed down the generations through millions of years, Dawkins argued, could be considered almost immortal. But they were still subject to the same fitness requirements that Darwin postulated.
Gene drives, in this scheme of survival of the fittest, are cheaters, card-sharks. They don’t play by the rules of evolution. They are genes that have figured out how to game the system, how to directly alter the molecular machinery of replication and spread more rapidly than chance would allow—even if there is a fitness cost to the organism in which they reside.
Some gene drives do this by carrying their own set of safecracking tools for hacking into DNA. Their toolkit contains a description of the genetic code to be replaced; scissors for cutting out that target sequence; and a new, altered sequence that will take its place. Once introduced into the DNA of an egg or sperm—the germline, which is passed on to offspring—the whole kit is bequeathed to the next generation. If a wild male mosquito mates with a female that has been equipped with a gene drive, any offspring will be altered to match the female, and the change will in time pass to all progeny.
The idea of using this kind of gene drive to manage ecosystems was first proposed in 2003 by Austin Burt, a professor at Imperial College London, who wrote a paper describing the possibility of using gene-cutting enzymes (meganucleases) to edit the genomes of wild mosquito populations in order to fight malaria. By inserting those gene cutters and instructions of what to cut, as well as replacement sequences, into germline cells, Burt hypothesized that one could push a change from altered mosquitoes into all their offspring, and so on, until virtually all the individuals in a species carried the change. In organisms with short life spans, changes could push through a population in a matter of months. Burt’s proposal was prescient, but the state-of-the-art gene-editing tools he proposed using were not good enough at the time to make development practical. That changed suddenly in the autumn of 2013, when Esvelt realized that incorporating Crispr-Cas9 (DNA cutting scissors that can be directed to cut any gene sequence specified) into the germline of an organism could make Burt’s vision viable. Andrea Smidler’s arrival from the lab of a leading mosquito geneticist was thus fortuitous.
From Technology to Ethics
But Esvelt realized that such a system would have application beyond mosquitoes—that gene drive biotechnology could change the way humanity interacts with the global environment. Scientists could make crops more nutritious or impervious to infection, and alter rodents and biting insects so they don’t transmit disease. Such drives are powerful: once released, they are designed to operate autonomously and, potentially, forever—barring a mutation in the target sequence—and to spread worldwide wherever the host organism lives.
And they could be built easily and inexpensively (with equipment costing less than $100,000, Esvelt estimates) in someone’s garage, by small groups or even an individual. They thus present a range of risks: that a bright but irresponsible teenager might alter the local housefly population so that it fluoresced; that an experimental drive designed to kill or alter a population of organisms might escape a lab before its use had been approved; that an approved and released drive might have unanticipated ecological impacts; that someone might use a gene drive for bioterrorism. In all these cases, Esvelt says, it would be possible to create a new gene drive to target and counter the effects of the undesirable drive. The real damage, he worries, would be to public opinion. “Which brings us to the further point: how are we going to deal with this technology? We have never before been faced with the capability to unilaterally alter the shared environment” with biological tools. Safety considerations are not trivial.
He and Church invited representatives of “every lab that had ever published a new method of using Crispr and fruit flies, every laboratory that ever published anything on DNA cutting and gene drives, including Austin Burt,” to develop safety protocols. “In all,” he recalls, “there were 27 of us who, over four months, hashed out guidelines…to ensure that no accidental release would happen.” Together with bioethicist Jeantine Lunshof, a visiting fellow who works in Church’s lab (“George is the only scientist I know,” says Esvelt, “who has a bioethicist working in his lab at all times”), they began publishing in 2014 a series of recommendations in Science for researchers working with gene drives—even before any such drives existed. (There are now four, all in laboratories: one in yeast, developed in Church’s lab; one in fruit flies; and two in mosquitoes).
In addition to multiple layers of biocontainment, they recommended conducting experiments only where the modified organism could not survive outside a controlled environment. Because Anopheles gambiae, the main carrier of the malaria parasite, requires constant high temperature and humidity, for example, working with them in temperate climates like Boston helps ensure that they could not reproduce if they escaped. The researchers also recommended intrinsic safeguards. A reversal drive can undo the effects of an earlier gene drive, if the original drive escapes the lab or fails to perform as desired. Immunization drives can “make a population resistant to a particular gene drive,” Church explains, protecting against an accidental release or unwanted spread of a drive. Another innovative safety mechanism he and his collaborators have proposed is to separate the Crispr guide RNA from the Cas9 cutting enzyme. Omitting the Cas9 scissors from the germline DNA would prevent editing of the genome in offspring, allowing researchers to safely test a genetic change without the risk that an accidental release might allow the alteration to spread through an entire species.
Church, who is among the few safety engineers in bioengineering, says it’s not common for biologists to suggest all the safety mechanisms before doing their first experiment. “It’s usually the other way around. They do a few experiments, maybe something goes wrong or it dawns on them” that something might go wrong. But with gene drives, he stresses, safety planning was the first step, because “there is no such thing as a limited release.” The second step has been testing those safety features in yeast, and the third will be testing them in mosquitoes like those in the insectary run by Catteruccia, who’s been deeply involved in these conversations. Testing might include trying to determine whether a gene drive could jump to another mosquito species, Church says. “You can compensate for the fact that your lab is small, relative to the wild, by putting them in closer proximity. Typically, species are isolated not just by their sexual preferences and morphology and chromosome behavior but also by opportunity.” The opportunity, he says, “you could force” in the lab.
“If you are talking about something that alters the shared environment,” Esvelt says, “you had better get it right.” He aims for a large collaborative effort to “figure out what can go wrong,” in order to “end up with the safest possible gene drive system ready for deployment. How can we in good conscience even begin those sorts of experiments without telling people what we are doing first?”
Alluring Applications
Despite these risks, Esvelt (now an assistant professor at MIT) believes that a carefully engineered gene drive might be far less harmful to the environment than traditional methods of controlling mosquitoes. Historically, the best way to combat malaria was to spray DDT. Until that insecticide’s toll, on birds in particular, became apparent in the last century, such chemical methods of control seemed elegant, and far less harmful to ecosystems, he says, than “the single most effective way we have right now to deal with malaria: Drain the swamp. Use bulldozers. Obviously that has tremendous ecological impact. You can imagine that a better way would involve learning to speak nature’s language. Gene drives present for the first time the possibility of targeting only one species, leaving the rest of an ecosystem intact.”
“Admittedly,” he acknowledges, “we don’t understand ecosystems. Ecology is more complicated than standard and molecular biology.” Evaluating a gene drive’s ecosystem effects, he explains, starts with understanding exactly what it is designed to do. Two mosquito gene-drive experiments published in late 2015, for example, use very different strategies to combat malaria, and thus yield very different outcomes. A U.K.-based group that includes Austin Burt has created what is known as a suppression drive in Anopheles gambiae, in line with Burt’s 2003 paper. Suppression drives affect an organism’s ability to reproduce; this one would render all female offspring of the species sterile. If released into the wild, it could lead to extinction, unless there were mutants that escaped. No one has ever mourned the loss of an individual mosquito—but killing an entire species might have consequences. Some mosquitoes may pollinate flowers; some provide food for dragonflies. On the other hand, the single study on this subject done in a region where malaria is endemic found that no known flower relied on the local Anopheles mosquitoes for more than 10 percent of its pollination needs, and no predator relied on them for more than 10 percent of its diet. Wiping out A. gambiae, then, might be acceptable when weighed against the health risk and devastating economic effects of malaria.
A second experimental mosquito gene drive, published in the fall of 2015 by researchers at the San Diego and Irvine campuses of the University of California, reengineers the genome of the Asian malaria-carrying species Anopheles stephensi so that it generates antibodies to the malaria parasite. This resistance drive confers immunity by ensuring that more than 98 percent of mosquitoes inherit malaria-resistant genes.
The differences between these malaria-fighting strategies are profound, Catteruccia explains. Suppression drives like Burt’s carry a risk of evasion and escape: if just one mosquito among millions carries a mutation in the target sequence, that individual will not be rendered infertile, and eventually its offspring will dominate the population, defeating the purpose of the drive. On the other hand, eradicating an entire species creates a different kind of risk: that something else will fill the empty ecological niche, with unknowable consequences. (For example, the eastern coyote, once a smallish, solitary hunter, has grown substantially larger, and at times hunts in packs for larger game—filling the niche extirpated timber wolves left behind.)
Resistance drives, in principle, are less disruptive to the ecosystem, because they leave a species largely intact. But the malaria parasite is itself notorious for its ability to adapt, so it, too, might “escape” the immune resistance of altered mosquitoes, if it could adapt quickly enough to remain fit in a changed environment. For that reason, Church says, it might be preferable to use both approaches—suppression and resistance—so the parasite has fewer chances to evolve.
How can scientists like Esvelt hope to predict the environmental consequences of a gene drive? “In exactly the same way as we do everything else in science,” he responds. “Through rigorous evaluation, hypothesis, testing—and repeating the cycle. If we do it transparently, and we invite people’s feedback on what we’re doing, then you have more heads looking at the problem. You are more likely to detect something that might have slipped by than if you were just working in a small team in a laboratory, as in conventional science.”
Andrea Smidler, working with Esvelt, Church, and Catteruccia, has been engaged in trying to create a mosquito gene drive of her own. She hopes to build one that would target and alter a single gene in more than one place. That way, a random mutation in any one of the gene drive’s target sequences would not disable the entire drive. At the same time, she hopes to prevent the risk of “escape,” through innovative means: by linking the drive to sequences in which a mutation of any kind would prove so costly to the mosquito’s fitness that it would not survive.
Gene drive technology will likely be ready for application, whether in mosquitoes or another species (perhaps the wild mice that carry the bacterium that causes Lyme disease), before the public fully understands the ramifications of taking such a step. That’s why Church, Esvelt, Catteruccia, and others—in parallel with their own work on safety testing and development—have emphasized the need for public engagement and discussion, not only in their own countries but worldwide. “There’s tremendous humanitarian need for a lot of these applications,” says Esvelt. “And the limiting factor may not be the time required for us to build a gene drive in the laboratory. It may be the time required for society to decide whether or not it should be used.”
And scientists, he adds, must accept the possibility that society could say no, halting gene-drive research entirely. “I, for one, would much rather be told ‘no’ at an early stage,” he says, “before I’ve invested a lot of time and effort working on [a project].”
Catteruccia notes one practical detail that should not be overlooked. Even if governments embrace gene-drive technology for its promise in a specific application, and the ramifications are fully and publicly debated, gene drives still require sexual reproduction to work. In the case of malaria, “Research in that area is really lagging,” she says, but it is “key to the success of this technology. Because you can have the fanciest technology on earth, the perfect gene drive, but if your lab mosquitoes can’t mate with wild mosquitoes, then it’s not going to work at all.”